Volume 2 - Year 2015 - Pages 11-20
DOI: 10.11159/jbb.2015.002
Isolation of Cellulolytic Organisms from the Gut Contents of Termites Native to Nepal and Their Utility in Saccharification and Fermentation of Lignocellulosic Biomass
Dinita Sharma1, Bishnu Joshi1,2, Megh Raj Bhatt1, Jarina Joshi1, Rajani Malla1, Tribikram Bhattarai1, Lakshmaiah Sreerama3,4
1Central Department of Biotechnology, Tribhuvan University, Kirtipur, Nepal
2National College, NIST, Kathmandu, Nepal
3Department of Chemistry, St Cloud State University, St Cloud, MN 56301, USA
4Department of Chemistry and Earth Sciences, Qatar University, Doha, Qatar
dinitasharma@gmail.com
Abstract - Lignocellulose is one of the most abundant renewable materials that could serve as a source for fermentable sugars. However, its commercial deployment is limited by the fact that lignocellulose is recalcitrant and requires heroic efforts to release sugars. Although very few, there are cellulose degrading organisms in nature that can efficiently degrade the cellulosic biomass and one among them is termite. Termites are responsible for mass turnover of the cellulosic biomass and they accomplish this task by harbouring a consortium of cellulolytic and lignolytic microorganisms. In the present study, we have isolated eight bacterial and five fungal cellulose degrading organisms from the gut of a termite native to Nepal via basal culture medium enrichment with filter paper as substrate. The isolated organisms were identified as Bacillus sp., Cellulomonas sp., Enterobacter sp., and Aspergillus sp. The Congo red screening assay for cellulase production showed the largest zone of hydrolysis (38 mm) for Aspergillus sp. The carboxymethyl cellulose assay revealed that the Bacillus sp (S3B8; 0.12+ 0.01 IU/ml obtained within96 hours) and Aspergillus sp. (S3F3; 0.07 +0.01 IU/ml obtained within 168 hours) were most efficient cellulase producers. The saccharification and fermentation of lignocellulosic biomass to ethanol was successfully achieved via culturing of selected bacterial [Bacillus (S3B8)] and fungal isolates [Aspergillus (S3F3)] and then fermentation with Saccharomyces cerevisiae (S2Y1). Further characterization of these organisms and optimization of fermentation of lignocellulosic biomass is ongoing.
Keywords: Cellulase, Saccharum spontaneum.
© Copyright 2015 Authors - This is an Open Access article published under the Creative Commons Attribution License terms. Unrestricted use, distribution, and reproduction in any medium are permitted, provided the original work is properly cited.
Date Received: 2014-12-30
Date Accepted: 2015-09-22
Date Published: 2015-09-29
1. Introduction
Generation of biofuels (ethanol) from sugars derived from lignocellulosic biomass is one of the challenging as well as a promising alternative to produce clean liquid fuels. The challenge comes from the fact that efficient and inexpensive bioprocessing configurations leading to commercially viable technologies are yet to be developed. It is promising because the lignocellulosic biomass is abundant in nature and these sources are renewable.
Lignocellulosic biomass is complex matrix with three major components, viz., lignin, cellulose (polymer of beta-glucose units) and hemicellulose (polymer of C5 sugars). More than 70% of the dry mass lignocellulose is made of sugar polymers. Cellulose is a chemically simpler substance containing repeating units of glucose, but complex structurally because of the presence of β-1,4-linkages (not easily susceptible to breakage) and its complexing with lignin and hemicellulose[1]. Breakdown of cellulose involves a set of hydrolytic enzymes including cellulases that are responsible for the depolymerization of cellulose into fermentable sugars. The three main types of enzymes responsible for the hydrolysis of cellulose are endoglucanases (EC 3.2.1.4), cellobiohydrolases or exoglucanases (EC 3.2.1.91) and β-glucosidases (EC3.2.1.21) [1, 2].Endoglucanases cut at random at internal amorphous sites in the cellulose polymer and generating oligosaccharides of various lengths [3]. Exoglucanase hydrolyzes the reducing and/or non-reducing ends of cellulose polymer liberating either glucose or cellobiose as major products. Finally, β-glucosidases hydrolyzes cellobiose to glucose. The cellulase enzyme production accounts for 40% of total cost in bioethanol synthesis [4]. To reduce cost of production, on site production of crude enzyme from indigenous microbes is more viable than commercial cellulase due to their reasonable cost (exclusion of enzyme purification step), high enzyme production capacity, etc. The reduction in this cost paves a cost effective way for ethanol production [5].
Termites are considered as the potent source of cellulase and laccase enzymes. They belong to the order Isoptera [6] and are the major consumers of the lignocellulosic biomass in tropical and subtropical ecosystems. It is estimated that termites ingest 50%-100% of the dead plant biomass in tropical ecosystems and dissimilate about 74-99% cellulose, 65-87% hemicelluloses part of lignocellulose they ingest [7]. Cellulolytic bacteria such as Acinetobacter, Pseudomonas, Staphylococcus and various species of Enterobacteriaceae and Bacillaceae families have been reported to be present in the termite gut [8].
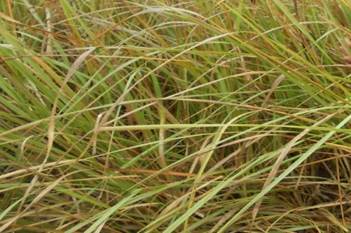
Among the lignocellulosic biomass, Saccharum spontaneum (Figure 1), a cultivar of switch grass, is a weed that commonly grows in the tropical countries such as Nepal. Its growth is extremely fast and its fermentable sugar content is very high, accordingly considered a potential source of fermentable sugars for the production of ethanol. S. spontaneum is believed to be a predecessor of S. officinarum [9]. The Saccharum genus contains thirteen species (US Department of Agriculture) and it is similar to the Miscanthus genus; it differs from the latter by the different disposition of the spikelets in the bloom and the rachis fragility [10]. The plant stem and outer sheath have high content of carbohydrate fraction in its cell wall (67.85% by wt.). In the past decade, there has been tremendous progress in the field of renewable energy procurement. With regard to ethanol in particular, the focus has been on its production by second generation processes [11, 12].
In fact, many countries are moving towards developing or have already developed technologies to exploit the potential of lignocellulosic materials for the production of bioethanol. This process generally involves pre-treatment and hydrolysis of lignocellulosic biomass to fermentable sugars followed by fermentation of such sugars to ethanol [13]. Achieving fermentable levels of sugars from lignocellulosic biomass requires relatively harsh pre-treatment processes, costly enzymatic process and design of different processing tanks for the saccharification, hydrolysis and fermentation (SHF) processes [14]. Overall, these processes increase production cost of the ethanol. Accordingly, our aim is to design ways to produce cellulosic ethanol in a cost effective manner using indigenous microbes isolated from gut of termites native to Nepal. In the present study, cellulolytic microorganisms have been screened for cellulase activity and further evaluated for ethanol production using pre-treated S. spontaneum as the biomass.
2. Materials and Methods
2. 1. Sample Collection and Processing
S. spontaneum was collected from Kirtipur and Rasuwa areas of Nepal. After locating the site of collection, the weedy biomass was collected in the clean and dry polythene bags. The samples were then transported to the biotechnology laboratory in Tribhuvan University, Nepal for further processing. The substrates were washed with tap water, if any adhering dirt were present. It is then dried, first at sun drying and then in hot air oven at the temperature of about 90˚C for 24 hours. The dried plant materials were then powdered by the combination of chipping and milling. The milled biomass is sieved to attain the particle size of about 3-4 mm.
2. 2. Isolation of Bacteria
Termite samples were collected from different places in Kathmandu, Nepal, in sterile bottles. They were fed with the woody materials collected from the sampling site. Ten termites were surface sterilized with 70% ethanol and the entire gut of the termite was picked out with the help of sterile tweezers and suspended in 1 mL 0.85% NaCl under sterile condition. The gut suspension (0.5 mL) was mixed with 4.5 ml of bacterial culture broth containing carboxymethylcellulose (CMC) and yeast extract 5% (w/v) and 0.2%tryptone (w/v), respectively (Medium-1). The mixture was incubated at 30˚C for 7 days for enrichment. After this incubation, 1 mL of the culture was spread on Medium-2 containing K2HPO4 (0.2 g/L),KH2PO4 (0.2 g/L), MgSO4(0.2 g/L), NaCl (0.2 g/L),NaNO3(1 g/L),CaCO3 (0.01 g/L),yeast extract (0.5 g/L),CMC (10 g/L),Agar(15 g/L), pH 7.0 and incubated for 24 hours at 30˚C [15]. The pure bacterial colonies were obtained after subsequent sub-culturing in nutrient agar.
2. 3. Screening for Cellulolytic Bacteria
The pure colonies of organisms were allowed to grow in 50 ml Medium-2 containing 0.2 g/L K2HPO4, 0.01 g/LMgSO4, 1g/L NaCl, 0.5 g/L NaNO3, 5% Yeast Extract and 10g/LCMC. The pH was maintained at 7 and cultures were incubated at 30˚C for 72 hours. At the end of this incubation 1 mL of the culture suspensions were centrifuged at 5000 rpm for 5 minutes and the pellets were re-suspended in fresh medium. Using new 1.5% Agar plates supplemented with 1% CMC and sterile cork-borer, 6mm wells were punched. The wells were filled with 100 μL of the culture re-suspension prepared above and the plates were incubated at 30˚C for 24 hours. At the end of this incubation, the plates were flooded with 0.1% Congo red for 15 minutes and de-stained with 1M NaCl solution for 15 minutes. The clear zones represented by CMC hydrolysis were measured.
2. 4. Morphological and Biochemical Characterization of Bacteria
The pure cellulolytic bacteria isolated by the techniques described above were further subjected to various morphological and biochemical tests for their identification. To determine cellular morphology, the bacteria were first stained with Gram's reagents, the characteristics were recorded using a compound microscope and compared to the characteristics described in Bergey's Manual of Systematic Bacteriology [16]. The biochemical tests performed include, triple iron sugar test, catalase activity, oxidase activity, nitrate test, oxidative fermentative test, citrate test, methyl red test and Voges Proskauer test. Motility tests were performed using standard methods. Acid and gas production after carbohydrate fermentation was detected by the visible change in colour from red to yellow using sugar utilization tests.
2. 5. Process Optimization of Maximum Cellulase Production
For the maximum production of cellulase enzyme by the isolated bacteria were determined different cultural conditions (optimal pH, temperature, time of incubation, carbon source, and substrate concentration) as described previously by Gautam et al.. [17]
2. 6. Carboxy Methyl Cellulose (CMC) Assay
The standard CMC assay as described by Ghose [18] was used for the quantification of the cellulase activity with some modifications. Briefly, to test the fungal cultures for cellulose activity, the substrate (1% CMC) was prepared in 0.05 M sodium citrate buffer (pH 5), whereas in the case of bacterial filtrates, the substrate (1% CMC) was prepared in 0.05 M sodium phosphate buffer (pH 7). The assay was performed at 50˚C for 30 minutes. Hydrolysis of CMC was terminated by the addition of di-nitrosalicylic acid (DNS) reagent (3.0 ml) and by subsequently placing the reaction tubes in a boiling water bath (100˚C) for 5.0 minutes. All samples (controls, blanks, and glucose standards) were treated identically. The samples were cooled and the absorbance was recorded at 540 nm against the blank. Glucose was used a standard and the equivalents of reducing sugar formed in the reactions was determined using a glucose standard curve. One unit of cellulase activity was expressed as 1μmole of glucose formed per mL enzyme per minute [18].
2. 7. Bioethanol Production
Ten gram of S. spontaneum (biomass) was transferred to 250 ml flask containing 100 ml 0.5 M ammonium hydroxide (NH4OH). It was thoroughly mixed for 5 minutes and incubated for 24 hours at 65˚C. The suspension was vacuum filtered to separate the solid residue. The solid residue was further washed with water until the filtrates were completely neutral and dried in hot-air oven at 105˚C for 6 hours. The oven-dried samples were stored in valve bags for further analysis and enzymatic hydrolysis. The first filtrate, post NH4OH treatment, was saved and analyzed for fermentable sugar level by DNS method [19].Pure cellulose, CMC and pre-treated S. spontaneum biomass were hydrolysed using the cellulase preparations from bacteria and fungi described above. The hydrolysis of substrates was performed in 0.05M sodium citrate buffer, pH 5 for fungal cellulase enzyme preparations and 0.05 M phosphate buffer of pH 7 for bacterial cellulase enzyme preparations. Hydrolysis was performed in 500 mL flasks containing 200 mL buffer, 50mL of cellulase enzyme preparation and 10 mg/mL of cellulose, CMC or Biomass as substrate at 500C in a shaker for 24 hours. The mixtures were centrifuged at 5,000 g for 10 minutes to separate the hydrolysates were first supplemented with filter-sterilized salt solution (0.4 g/L KH2PO4, 0.02 g/L MgSO4, 0.05 g/L CaCO3, and 0.01 g/L NaCl) and then fermented using S. cerevisae. At the end of this incubation, the culture broth was tested for alcohol production using the K2Cr2O7 reagent test [20].
3. Results and Discussion
The morphological and biochemical characteristics of eight cellulase-producing microbial isolates are described in Tables 1 and 2. These isolates have been identified to be Bacillus sp, Cellulomonas sp., Enterobacter sp., Aspergillus sp. and these findings are consistent with the findings reported by other workers in the field [21-23].
Table 1. Colony morphology of cellulolytic bacteria isolated from termite gut contents.
SN |
Sample |
Colour |
Shape |
Size(mm) |
Elevation |
Opacity |
1. |
S1B1 |
Creamy white |
Oval |
1.5-2 |
Convex |
Opaque |
2. |
S1B2 |
Creamy white |
Irregular |
3-4 |
Convex |
Opaque |
3. |
S1B5 |
White |
Oval |
3-3.5 |
Flat |
Opaque |
4. |
S2B1 |
Creamy white |
Oval |
3 |
Convex |
Opaque |
5. |
S2B5 |
Creamy white |
Circular |
1.-1.5 |
Convex |
Opaque |
6. |
S3B3 |
White |
Irregular |
1-2 |
Convex |
Opaque |
7. |
S3B6 |
Creamy white |
Circular |
1.5- 2 |
convex |
Opaque |
8. |
S3B8 |
Creamy white |
Irregular |
3-4 |
Convex |
Opaque |
3. 1. Isolation and Screening of Cellulolytic Microorganisms by Plate Staining Method
Among the thirty isolates (both bacterial and fungi),eight bacterial isolates (S1B1, S1B2, S1B5, S2B1, S2B5, S3B3, S3B6, S3B8) and five fungal isolates(S2F1,S2F2, S3F3, S1F1, S1F2) exhibit varying levels of cellulolytic activity as judged by plate staining methods (Table 3). An example of the appearance of the clear zone around the colonies after staining the plates with Congo has been shown in Figure 2. Among the fungal strains, S3F3 showed the largest zone of hydrolysis of 38 mm and among the bacterial isolates, S3B8 showed largest zone of hydrolysis of 32 mm. The clear zone among all isolates ranged from 13 to 38 mm (Table 3). It is important to note that the plate-screening method is not quantitative and the correlation between enzyme activity and colony to clear zone ratio is considered poor [24]. The colonies showing highest halo zones were picked from replica plates (S3B8 and S3F3) and further screened cellulose production in liquid medium using CMC as substrate. The crude preparations of S3B8 bacterial isolate and S3F3 fungal isolate showed 0.07 IU/mL and 0.08 IU/mL cellulolytic activity, respectively. Partially purified fractions (ammonium sulphate precipitation) of S3B3 bacterial isolate showed and enzyme activity of 0.12 IU/mL and whereas the S3F3 fungal isolate showed 0.2 IU/mL.
Table 2. Biochemical tests of different bacteria isolated from the termite gut.
SN. |
Test Sample |
TSI |
Citrate |
Sulphur |
Indole |
Motility |
Urease |
OF |
MR |
VP |
Nitrate |
Catalase |
Oxidase |
Gram stain |
1 |
S1B1 |
Y/R G + H2S- |
- |
- |
- |
+ |
- |
F |
_ |
+ |
+ |
+ |
- |
+ , Rod |
2 |
S1B2 |
Y/Y G - H2S- |
- |
- |
- |
? |
- |
F |
- |
+ |
- |
+ |
- |
+ ,Single rod |
3 |
S2B1 |
Y/Y G + H2S- |
+ |
- |
- |
- |
- |
F |
- |
+ |
+ |
+ |
- |
-,Rod |
4 |
S2B4 |
Y/Y G - H2S- |
+ |
- |
+ |
+ |
- |
O |
- |
+ |
+ |
+ |
- |
- ,Rod /cocci |
5 |
S2B5 |
Y/RG - H2S- |
+ |
- |
- |
+ |
- |
F |
+ |
_ |
+ |
+ |
- |
+ ,Single rod |
6 |
S3B3 |
Y/R G+ H2S- |
- |
- |
- |
? |
- |
F |
- |
+ |
_ |
+ |
- |
+ ,Single rod |
7 |
S3B6 |
Y/Y G- H2S |
- |
- |
- |
+ |
- |
F |
- |
+ |
+ |
+ |
- |
+, Rod in chain |
8 |
S3B8 |
Y/RG + H2S- |
- |
- |
- |
+ |
- |
F |
- |
+ |
+ |
+ |
- |
+ ,Rod in chain |
*Note: '+' - positive result, '-' - negative result, TSI: triple sugar iron test, OF: oxidative/fermentative test, MR: methyl red test, VP: Voges Proskauer test.
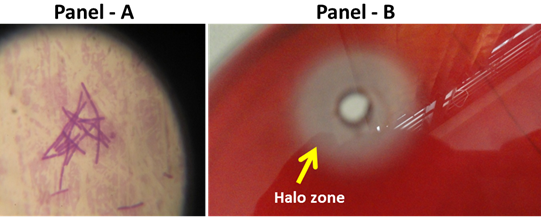
3. 2. Optimization of Cultural and Nutritional Conditions for Cellulase Production
The isolated bacterial strain Bacillus and fungal strain Aspergillus had the ability to utilize various additional carbon sources. It was observed that CMC gave maximum cellulase activity at 1.5% concentration i.e. (0.08 + 0.001 IU/mL) for bacterial cellulase and (0.12 + 0.01 IU/mL) for fungal cellulose when compared to other carbon sources (Figure 3). These results are in agreement with work of Nirjane et al. [25]. Nirjane et al. [24] have observed that Bacillus alcalophilus S39 and Bacillus amyloliquefaciens C2, results in increase in enzyme activity when CMC was used as a substrate. A medium containing glucose as a carbon source presented the minimum activity because cellulase production is directly proportional to the crystallinity of the biomass from which it is produced, i.e. the higher the crystallinity, the better is the yield of cellulase. This is because the glucose in the medium acts as the catabolite repressor of the cellulase enzyme, and thus reduces its activity.
Table 3. Zone of hydrolysis shown by pure bacteria and fungi in the Congo red assay.
S.N. |
Sample (Bacteria) |
Diameter of halo zone (mm) |
S.N. |
Sample (Bacteria) |
Diameter of halo zone (mm) |
S.N. |
Sample (Fungi) |
Diameter of halo zone (mm) |
1 |
S1B1 |
22 |
12 |
S2B7 |
11 |
1 |
S1F1 |
20 |
2 |
S1B2 |
17 |
13 |
S3B1 |
08 |
2 |
S1F2 |
18 |
3 |
S1B3 |
14 |
14 |
S3B2 |
13 |
3 |
S1F3 |
13 |
4 |
S1B4 |
X |
15 |
S3B3 |
17 |
4 |
S2F1 |
20 |
5 |
S1B5 |
29 |
16 |
S3B4 |
10 |
5 |
S2F2 |
35 |
6 |
S2B1 |
20 |
17 |
S3B5 |
15 |
6 |
S2F3 |
22 |
7 |
S2B2 |
X |
18 |
S3B6 |
23 |
7 |
S3F1 |
X |
8 |
S2B3 |
X |
19 |
S3B7 |
14 |
8 |
S3F2 |
X |
9 |
S2B4 |
13 |
20 |
S3B8 |
32 |
9 |
S3F3 |
38 |
10 |
S2B5 |
19 |
21 |
S3B9 |
13 |
|||
11 |
S2B6 |
X* |
|
|
|
*Note X: No zone of hydrolysis
In this research, the enzyme production increased with an increase in the concentration of cellulose up to 1.5% (W/V). Further increase in cellulose concentration beyond 1.5% did not result in proportionate increase in the activity. Production of cellulase by bacteria Bacillius and fungi Aspergillus in response to CMC concentration followed the same trend. The results clearly showed that supplementation of cellulose at 1.5% were optimal for cellulase production, however a study conducted by Abou-Taleb et al. [26] showed that 1% CMC gave the highest activity for Bacillus strains. Given the above, the increase in cellulase production is dependent on the availability of substrate in the medium; while a decrease in production beyond optimum concentration can be explained as a result of an inhibitory effect of accumulated cellobiose and cellodextrins of low degree of polymerization in the growth medium. The medium used also contains different minerals apart from carbon source. An increase in the concentration of substrate may also increase in concentration of nutrients that may adversely affect the cell growth which in turn may also affect enzyme production.
3. 3. Effect of pH
The optimal pH for endoglucanase activity is found to be at pH 7 (0.08 + 0.01IU/mL) for bacterial cellulase and 5.5 (0.10 + 0.01IU/mL) for fungal cellulase. Increasing or decreasing the pH beyond this resulted in decline in enzyme activity (Figure 4). Our results are in agreements with previous findings [27]. Bacillus amyloliquefaciens C2 gradually increase with pH with an optimum at pH 7.0. [26]. The pH dependence of cellulolytic enzymes production among the fungal species is also similar [25]. For example, maximum production of β -glucosidase in Aspergillus terreus cultures was found in the pH range of 4.0-5.5 [29].
3. 4. Effect of Incubation Temperature
Cellulolytic enzyme production is also temperature dependent. In our experiments, the Bacillus S3B8 bacterial isolate exhibited maximum enzyme production at 38˚C; 0.077 + 0.003 IU/mL and fungal isolate Aspergillus S3F3 exhibited maximum enzyme production at 28˚C; 0.095 + 0.004 IU/mL (Figure 5). It is believed that the temperature influences the secretion of extracellular enzyme by possibly changing/or altering the physical properties of the cell membrane. Immanuel et al. [28] recorded maximum endoglucanase activity in Cellulomonas, Bacillus and Micrococcus species at 40° C and at a neutral pH.
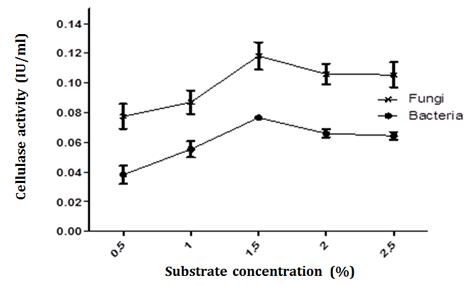
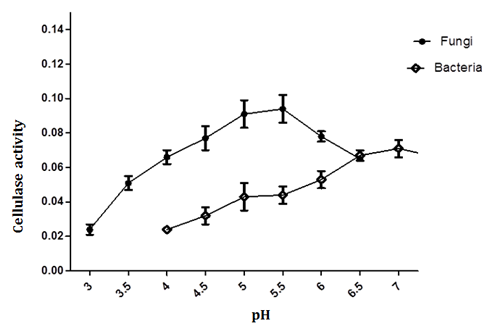
3. 5. Bioethanol Production
The experimental setup for saccharification and fermentation involved saccharification by bacterial isolate Bacillus S3B8 and the fermentation via Saccharomyces cerevisiae. The optimum temperature of the yeast was 28˚C for ethanol production. Saccharomyces cerevisiae (S2Y1) produced 4.9 g/L ethanol from CMC, 4.1 g/L from cellulose, and 3.6g/L from S. Spontaneum pre-treated with (0.5 M NH4OH) and hydrolysed substrate using cellulase obtained from Bacillus (S3B8) strain. Similarly for the fungal Aspergillus species (S3F3) fermentation was carried out with 15 ml of filtrate. Ethanol concentrations of the fermentations were determined at 6, 12, 18, 24 and 30 hours after inoculation. The mean ethanol concentration was found to be 4.32 g/L after 30 hour of the incubation.
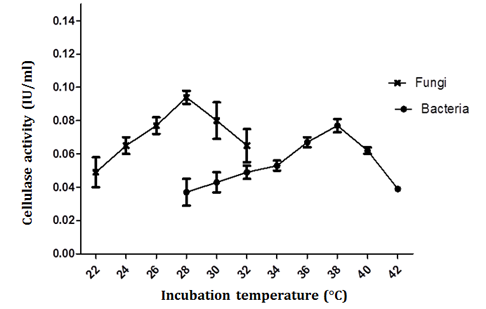
4. Conclusion
Lignocellulosic biomass has several advantages over conventional sugar- and starch-based raw materials and it has been projected to be one of the main sources of bioethanol in the near future. The aim of the present work was to isolate and identify a high cellulase producer from gut of termite. The production of ethanol from any lignocellulosic biomass involves feedstock pre-treatment, enzymatic separation, fermentation and ethanol recovery. S. spontaneum can be the potential source of feed stock for the bioethanol production, since it has high cellulose content and yields maximum fermentable sugars. The alkaline pre-treatment followed by saccharification and fermentation technique has been employed for bioethanol production in the present study. To further decrease the cost of step in biomass conversion to ethanol, it is essential to minimize the sugar losses and to increase the solid concentration as high as possible. Similarly, different commercialization strategies such as cost of cellulase enzyme, enzyme specific activity, and optimum enzyme loadings; concentration of fermentable sugars obtained after pre-treatment and enzymatic hydrolysis; fermentation configuration and process strategy; robust and hyper-producing (recombinant) microbial strains; capability to produce cellulases, hexose and pentose utilization; high ethanol tolerance; in situ ethanol separation to decrease ethanol inhibition; process water economy; commercial exploitation of the side products that are formed/can be produced during ethanolic fermentation; and integration of the ethanol plant with a power generation should be addressed.
References
[1] L. R. Lynd, P. J. Weimer, W. H. Van Zyl and I. S. Pretorius, "Microbial cellulose utilization: fundamentals and biotechnology," Microbiology and molecular biology reviews, vol. 66, no. 3, pp. 506-577, 2002. View Article
[2] L. R. Lynd, W. H. v. Zyl, J. E. McBride and M. Laser, "Consolidated bioprocessing of cellulosic biomass: an update," Current Opinion in Biotechnology, vol. 16, no. 5, pp. 577-583, 2005. View Article
[3] K. S. Siddiqui, A. M. Shemsi, M. A. Anwar, M. H. Rashid and M. I. Rajoka, "Partial and complete alteration of surface charges of carboxymethylcellulase by chemical modification: thermostabilization in water-miscible organic solvent," Enzyme and Microbial Technology, vol. 24, no. 8-9, pp. 599-608, 1999. View Article
[4] K. A. Gray, L. Zhao and M. Emptage, "Bioethanol," Current opinion in chemical biology, vol. 10, no. 2, pp. 141-146, 2006. View Article
[5] S.-T. Yang and S. Yang, "Bioprocessing-from Biotechnology to Biorefinery," Bioprocessing for Value-Added Products from Renewable Resources: New Technologies and Applications, Elsevier, Chapter 1, 2007. View Book
[6] T. Matsui, G. Tokuda and N. Shinzato, "Termites as functional gene resources," Recent Patents on Biotechnology, vol. 3, no. 1, pp. 10-18, 2009. View Article
[7] M. Ohkuma, "Termite symbiotic systems: efficient bio-recycling of lignocellulose," Applied microbiology and biotechnology, vol. 61, no. 1, pp. 1-9, 2003. View Article
[8] Z. Pourramezan, G. Ghezelbash, B. Romani, S. Ziaei and A. Hedayatkhah, "Screening and identification of newly isolated cellulose-degrading bacteria from the gut of xylophagous termite Microcerotermes diversus (Silvestri)," Microbiology, vol. 81, no. 6, pp. 736-742, 2012. View Article
[9] T. C. Sastry and K. Kavathekar, Plants for reclamation of wastelands. Publications & Information Directorate, Council of Scientific & Industrial Research, 1990. View Book
[10] L. Scally, T. Hodkinson and M. Jones, "Origins and Taxonomy of Miscanthus," Miscanthus for Energy and Fibre, London, UK: Earthscan, Capter 1, 2001. View Book
[11] A. Demirbas, "Products from lignocellulosic materials via degradation processes," Energy Sources, Part A: Recovery, Utilization, and Environmental Effects, vol. 30, no. 1, pp. 27-37, 2007. View Article
[12] A. K. Chandel and O. V. Singh, "Weedy lignocellulosic feedstock and microbial metabolic engineering: advancing the generation of 'Biofuel'," Applied microbiology and biotechnology, vol. 89, no. 5, pp. 1289-1303, 2011. View Article
[13] B. Joshi, M. R. Bhatt, D. Sharma, J. Joshi, R. Malla and L. Sreerama, "Lignocellulosic ethanol production: current practices and recent developments," Biotechnology and Molecular Biology Reviews, vol. 6, no. 8, pp. 172-182, 2011. View Article
[14] S. Banerjee, S. Mudliar, R. Sen, B. Giri, D. Satpute, T. Chakrabarti and R. Pandey, "Commercializing lignocellulosic bioethanol: technology bottlenecks and possible remedies," Biofuels, Bioproducts and Biorefining, vol. 4, no. 1, pp. 77-93, 2010. View Article
[15] M. Ramin, A. Alimon and N. Abdullah, "Identification of cellulolytic bacteria isolated from the termite Coptotermes curvignathus (holmgren)," Journal of Rapid Methods & Automation in Microbiology, vol. 17, no. 1, pp. 103-116, 2009. View Article
[16] W. B. Whitman, M. Goodfellow, P. Kämpfer, H.-J. Busse, M. E. Trujillo, W. Ludwig, K.-i. Suzuki and A. Parte, Bergey's manual® of systematic bacteriology. Springer, vol. 5, 2012. View Book
[17] S. Gautam, P. Bundela, A. Pandey, J. Khan, M. Awasthi and S. Sarsaiya, "Optimization for the production of cellulase enzyme from municipal solid waste residue by two novel cellulolytic fungi," Biotechnology research international, vol. 2011, 2011. View Article
[18] T. Ghose, "Measurement of cellulase activities," Pure and Applied Chemistry, vol. 59, no. 2, pp. 257-268, 1987. View Article
[19] G. L. Miller, "Use of dinitrosalicylic acid reagent for determination of reducing sugar," Analytical chemistry, vol. 31, no. 3, pp. 426-428, 1959. View Article
[20] S. Poznanski, "The analysis of mixtures of ethyl alcohol, ethyl acetate, acetic acid and water," Journal of the American Chemical Society, vol. 50, no. 4, pp. 981-988, 1928. View Article
[21] P. Chantawannakul, A. Oncharoen, K. Klanbut, E. Chukeatirote and S. Lumyong, "Characterization of proteases of Bacillus subtilis strain 38 isolated from traditionally fermented soybean in Northern Thailand," Sci Asia, vol. 28, pp. 241-5, 2002. View Article
[22] W. Win, Z. Lianhui, L. Dog, W. Yong, Z. Zhenshan and M. Zhihuai, "Conditions study of cellulose and acid protease production during the process of solid state fermentation of flaxseed meal," American Society of Agriculture and Biological Engin, vol. 34, no. 6, pp. 45-51, 2008.
[23] S. Gupta, "Optimization of Cellulase Production from Bacteria Isolated from Soil," ISRN Biotechnology, vol. 2013, 2013. View Article
[24] M. Maki, K. T. Leung and W. Qin, "The prospects of cellulase-producing bacteria for the bioconversion of lignocellulosic biomass," International Journal of Biological Sciences, vol. 5, no. 5, pp. 500, 2009. View Article
[25] A. P. Niranjane, P. Madhou and T. W. Stevenson, "The effect of carbohydrate carbon sources on the production of cellulase by Phlebia gigantea," Enzyme and Microbial Technology, vol. 40, no. 6, pp. 1464-1468, 2007. View Article
[26] K. A. Abou-Taleb, W. Mashhoor, S. A. Nasr, M. Sharaf and H. H. Abdel-Azeem, "Nutritional and environmental factors affecting cellulase production by two strains of cellulolytic Bacilli," Australian Journal of Basic and Applied Sciences, vol. 3, no. 3, pp. 2429-2436, 2009. View Article
[27] O. Fagade and O. Bamigboye, "Effect of cultural conditions on the cellulase activity of bacteria species isolated from degrading corn cob," Archives of Applied Science Research, vol. 4, no. 6, pp. 2540-2545, 2012. View Article
[28] G. Immanuel, R. Dhanusha, P. Prema and A. Palavesam, "Effect of different growth parameters on endoglucanase enzyme activity by bacteria isolated from coir retting effluents of estuarine environment," International Journal of Environmental Science & Technology, vol. 3, no. 1, pp. 25-34, 2006. View Article
[29] S. Pushalkar, K. Rao and K. Menon, "Production of β-glucosidase by Aspergillus terreus," Current microbiology, vol. 30, no. 5, pp. 255-258, 1995. View Article